Ureilite
Polymict
Shallow regolithMixture of unconsolidated rocky fragments, soil, dust and other fine granular particles blanketing the surface of a body lacking an atmosphere. Regolith is the product of "gardening" by repeated meteorite impacts, and thermal processes (such as repeated heating and cooling cycles). Click on Term to Read More fragmental brecciaWork in Progress ... A rock that is a mechanical mixture of different minerals and/or rock fragments (clasts). A breccia may also be distinguished by the origin of its clasts: (monomict breccia: monogenetic or monolithologic, and polymict breccia: polygenetic or polylithologic). The proportions of these fragments within the unbrecciated material Click on Term to Read More
click on image for a magnified view
Fell October 7, 2008
20° 43.04′ N., 32° 30.58′ E.
In 2008, October 6 at 5:46 A.M., while on duty at the Catalina Sky Survey at Mount Lemmon Observatory, Tucson, Arizona, Richard Kowalski discovered a small asteroid with a diameter of ~4 m, quickly designated 2008 TC3, that was calculated to have a near certainty of impacting Earth in 20 hours. This would be the first time an asteroid was spotted before it impacted Earth, tracked and then had its impact location and time predicted all within a matter of hours from when it was discovered. Those calculations indicated that the impact would occur in northern Sudan at 5:46 A.M. local time. Steve Chesley at JPL was able to calculate the time and location of the fallMeteorite seen to fall. Such meteorites are usually collected soon after falling and are not affected by terrestrial weathering (Weathering = 0). Beginning in 2014 (date needs confirmation), the NomComm adopted the use of the terms "probable fall" and "confirmed fall" to provide better insight into the meteorite's history. If Click on Term to Read More within 2 seconds and 1 mile of the actual location! After notification was made to several government agencies (e.g., NASA/JPL Near-Earth Object Program Office, the Jet Propulsion Laboratory, the Minor Planet Center, Sandia National Laboratories), observations of the asteroid began at many observatories around the world. Telescopic and light curve observations revealed that the asteroid had acquired both a rapid spin rate of 100 seconds and a tumbling motion (Sánchez and Scheeres, 2014).

Asteroid 2008 TC3 prior to atmospheric entry.
Credit: M. Kozubal & Ron Dantowitz—Clay Center Observatory
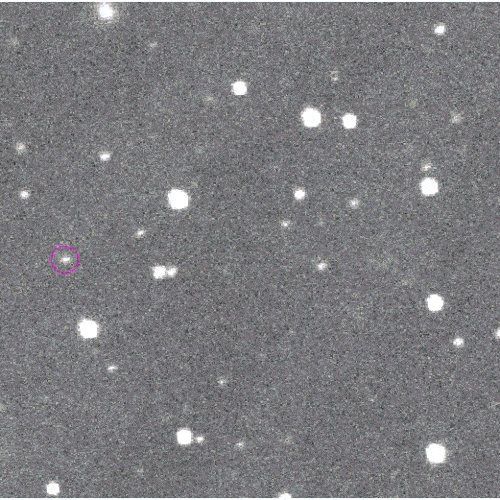
A sequence of images of asteroid 2008 TC3 taken at Mount Lemmon Observatory.
Credit: Richard Kowalski and Ed Beshore, Catalina Sky Survey
From the cockpit of Air France, KLM flight 592, pilot Ron de Poorter received a message from Jacob Kuiper of the Royal Netherlands Meteorological Institute regarding the possibility of observing the atmospheric entry of asteroid 2008 TC3. The crew witnessed the entrance as a flash of light below the horizon. It was later calculated that the entry velocity of the asteroid was 12.78 km per second at a height of 100 km (Welten et al., 2010), with an entry angle of 20°.
US and European satellites tracked the incoming meteorHow long Sonic booms Of the several 10s of tons of cosmic material entering Earth's atmosphere each day, only about one ton reaches the surface. An object's chance of survival depends on its initial mass, speed and angle of entry, and friability (tendency to break up). Micrometeoroids radiate heat so Click on Term to Read More from an altitude of 65 km and obtained photos. At the same time, Sudanese villagers were eyewitness to the fireballA fireball is another term for a very bright meteor, generally brighter than magnitude -4, which is about the same magnitude of the planet Venus as seen in the morning or evening sky. A bolide is a special type of fireball which explodes in a bright terminal flash at its end, often with visible fragmentation. Click on Term to Read More as it experienced major ablationGradual removal of the successive surface layers of a material through various processes. • The gradual removal and loss of meteoritic material by heating and vaporization as the meteoroid experiences frictional melting during its passage through the atmosphere. The resulting plasma ablates the meteor and, in cases where a meteor Click on Term to Read More at an altitude between 44 and 35 km, and then underwent a catastrophic disruption at 37 km. Infrasound monitoring stations in Kenya detected airwaves equivalent to 1.1–2.1 kilotons of TNT, about one-tenth the size of the Hiroshima atomic bomb. The meteor was comparable to a PE type IIIa/b fireball, akin to a fragile cometary mass (Ceplecha et al., 1998).

Artist’s conception of the pre-impact shape of asteroid 2008 TC3
based on actual observations as shown by the horizontal line at the top.
Photo Credit: P. Scheirich, P. Jenniskens
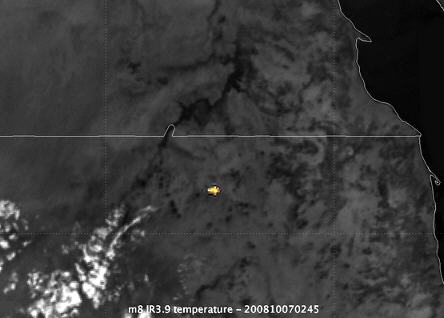
Real-time IR and visual composite image of 2008 TC3 final detonation ~37 km in altitude.
Image from the European Space Agency’s Meteosat 8 weather satelliteBody in orbit (such as a moon) around another larger body (such as a planet or star). Click on Term to Read More.
(http://asima.seti.org/2008TC3/images/Meteosat8impact.jpg)

Contrail of 2008 TC3 at dawn distorted by upper atmosheric winds.
Photo Credit: Mohamed Elhassan Abdelatif Mahir (Noub NGO),
Dr. Muawia H. Shaddad (Univ. Khartoum),
Dr. Peter Jenniskens (SETI Institute/NASA Ames)

Ground path of the meteoroidSmall rocky or metallic object in orbit around the Sun (or another star). over Sudan.
Red line is the object’s path, terminating where it would have hit the ground.
Green line is the infrasound detection of the explosion.
Orange crosshairs mark the fireball location according to Meteosat 8 IR data.
Map of Sudan: CIA Factbook. Graphic overlay: George W. Herbert, 10-07-2008.
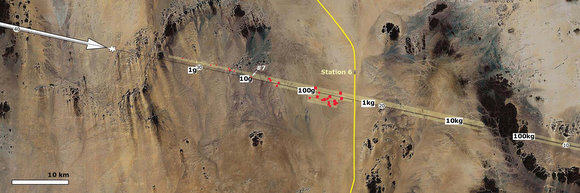
Ground path indicating the location of recovered meteorites (red dots).
On December 6, 2008, Peter Jenniskens of the SETI Institute in California flew to the Nubian Desert, Sudan to begin a search for meteorites from the fall. He and Muawia Shaddad, an astronomer from the University of Khartoum, Sudan, along with 45 university students and staff, enlisted the help of eyewitnesses to pinpoint the likely fall location. After an organized search, a student, Mohammed Alameen, found the first fragment—a 4.4 g fragment from the first meteoriteWork in progress. A solid natural object reaching a planet’s surface from interplanetary space. Solid portion of a meteoroid that survives its fall to Earth, or some other body. Meteorites are classified as stony meteorites, iron meteorites, and stony-iron meteorites. These groups are further divided according to their mineralogy and Click on Term to Read More recovered following its detection as an asteroid in space. The nearest identifiable landmark was Station 6 along the railroad between Wadi Halfa and Abu Hamad, and so the meteorite was named Almahata Sitta, Arabic for Station 6. Over the next few weeks repeated field expeditions yielded over 600 to 700 centimeter-sized samples of a brecciated meteorite having a combined weight of 10.7 kg, part of an estimated total fall of ~40 kg (Shaddad et al., 2011) within a strewnfield measuring about 28 × 5 km. Succeeding searches yielded additional specimens which were analyzed and classified at the University of Münster.
Portions of the preceding account were gleaned from Nature News Feature, vol. 458, 26 March 2009, by Roberta Kwok, and from ‘Asteroid 2008 TC3 and the Fall of Almahata Sitta, a Unique Meteorite Breccia’, Cyrena Goodrich et al., Elements, vol. 10 (2014).
Initially, samples of the Alamahta Sitta meteorite were sent to NASA’s Johnson Space Center in Houston (Zolensky) and Carnegie Institution of Washington (Steele) for analysis and classification. The meteorite was determined to be a polymict ureilite exhibiting anomalous features in some samples, such as low olivine-to-pyroxene ratio (a pigeonite–olivineGroup of silicate minerals, (Mg,Fe)2SiO4, with the compositional endpoints of forsterite (Mg2SiO4) and fayalite (Fe2SiO4). Olivine is commonly found in all chondrites within both the matrix and chondrules, achondrites including most primitive achondrites and some evolved achondrites, in pallasites as large yellow-green crystals (brown when terrestrialized), in the silicate portion Click on Term to Read More ureilite), wide ranging silicateThe most abundant group of minerals in Earth's crust, the structure of silicates are dominated by the silica tetrahedron, SiO44-, with metal ions occurring between tetrahedra). The mesodesmic bonds of the silicon tetrahedron allow extensive polymerization and silicates are classified according to the amount of linking that occurs between the compositions, large-sized pores with friability, fine-grained texture, large silicate aggregates, and large carbonaceous aggregates that have experienced higher heating than those in any other ureilite. Some augite-bearing ureilite samples are thought to contain a late shock-melt component. Almahata Sitta is a fragmental breccia composed of various ureilite lithologies (perhaps ten identified), which have been resolved into four general types (Goodrich et al., 2015): 1) coarse-grained, compact, olivine–pyroxene, with petrographic variability; 2) fine-grained, highly porous, highly reduced, with features of high temperature shock metamorphism; 3) metal+sulfide-rich with enclosed ureilitic silicates; 4) feldspar-rich, igneous-textured andesite. Incorporated mineral fragments in Alamahta Sitta include polycrystalline olivine, pigeonite, low-Ca pyroxene, carbon-rich aggregates, kamacite, and troilite.
The fine-grained lithology of Alamahta Sitta has significant porosityThe volume percentage of a rock that consists of void space. Vesicular porosity is a type of porosity resulting from the presence of vesicles, or gas bubbles, in igneous rock such as the pumice presented here. Vesicular porosity is very rare in meteorites and is often associated with slag, one Click on Term to Read More (~20%) comprising a 3-D network. The olivine crystals lining the pore walls were formed by vapor deposition processes prior to re-accretion into the daughter asteroid, 2008 TC3. Mosaicism, foliation, and the presence of diamonds in fine-grained samples attest to high temperature shock metamorphism, which likely occurred during re-accretion. Coarse-grained samples are only weakly shocked (S2–3). The occurrence of significant porosity led some to conclude that the meteorite was spalled from relatively unconsolidated material existing in the outer layers (shallow regolith) of the daughter asteroid. This asteroid was known through pre- and post-impact spectral data to have been an F-class asteroid in Tholen taxonomy, measuring ~4.1 m in diameter and having a long axis measuring 6.7 (±0.8) m. This is a class of asteroids located at ~2.45 AU located near the 3:1 mean motion resonance (signifying the ratio between the orbital period of Jupiter and that of an asteroid).
The presence of graphiteOpaque form of carbon (C) found in some iron and ordinary chondrites and in ureilite meteorites. Each C atom is bonded to three others in a plane composed of fused hexagonal rings, just like those in aromatic hydrocarbons. The two known forms of graphite, α (hexagonal) and β (rhombohedral), have Click on Term to Read More and microdiamonds in Alamahta Sitta have also been verified, but the diamondOne of the naturally occurring forms of carbon found in meteorites. Each C atom is bonded through covalent sp3 hydrid orbitals to four others. The strength of the C-C bonds makes diamond the hardest naturally occurring substance (according to the Mohs scale) in terms of resistance to scratching. There are Click on Term to Read More exhibits Raman spectra that are distinct from those of other brecciated and unbrecciated ureilites (Ross et al., 2010, 2011); nevertheless, the diamond lies within the spectral range of unbrecciated ureilites. The measured diamond strain values in Almahata Sitta are higher than those in other ureilites, indicating it experienced either greater shock (~6–15 GPa) or less annealing. Diamond and/or lonsdaleiteHexagonal polymorph of carbon (C) that forms from meteoric graphite during impact. The immense heat and stress of the impact transforms the graphite into diamond, but retains graphite's hexagonal crystal lattice (below). Lonsdaleite was first identified from the Canyon Diablo meteorite at Barringer Crater (also known as Meteor Crater) in Click on Term to Read More formed from shocked graphite on the parent bodyThe body from which a meteorite or meteoroid was derived prior to its ejection. Some parent bodies were destroyed early in the formation of our Solar System, while others like the asteroid 4-Vesta and Mars are still observable today. Click on Term to Read More is attested by the presence of high-pressure, compressed graphite phases. The predominance of crystalline graphite accompanying diamond leads to the conclusion that shock is likely the major cause of diamond synthesis in ureilites (Ross et al., 2011). Diamond is also considered to have formed by chemical vapor depositionMethod for growing solids in which a gaseous precursor (containing fragments of the desired solid) is decomposed and deposited onto a desired surface. Chemical Vapor Deposition (CVD) is one of the most powerful synthetic methods in material science due to its remarkable flexibility. A variety of surfaces can be coated, Click on Term to Read More (CVD) in the solar nebulaThe primitive gas and dust cloud around the Sun from which planetary materials formed., a process which may be inferred by its N-isotopic composition. In addition, single crystal diamonds measuring at least 40 µm have been identified in coarse-grained ureilite phases of Almahata Sitta, which were also likely formed by the CVD process (Miyahara et al., 2012, 2015). The occurrence of diamond formed by CVD is also supported by an alternative smelting mechanism involving methane, which has been posited by Langendam and Tomkins (2012) to explain the presence of smelting within fractures and discontinuous smelting at grain boundaries.
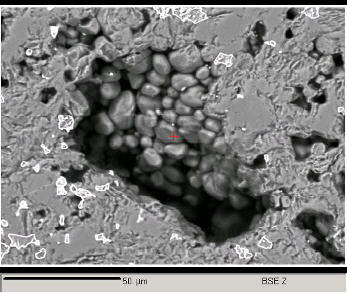
Pore wall lined with crystalline olivine produced by vapor deposition.
Photo Credit: M.E.Zolensky et al., 41st LPSC, #2306 (2010)
Nevertheless, large diamond crystallite assemblages have been identified in a low-shock lithology from the coarse-grained ureilite AhS MS-170. These diamonds exhibit almost identical crystallographic orientation and originally constituted a large (~100 µm) single-crystal diamond which had been fragmented during a shock-induced graphitization process—back transformation of diamond at low-pressure and high-temperature (Nabiei et al., 2018). Evidence for this scenario exists as inclusionFragment of foreign (xeno-) material enclosed within the primary matrix of a rock or meteorite. Click on Term to Read More trails consisting of nm-scale sulfide (plus kamaciteMore common than taenite, both taenite and kamacite are Ni-Fe alloys found in iron meteorites. Kamacite, α-(Fe,Ni), contains 4-7.5 wt% Ni, and forms large body-centered cubic crystals that appear like broad bands or beam-like structures on the etched surface of a meteorite; its name is derived from the Greek word Click on Term to Read More and schreibersiteNi-Fe phosphide mineral, (Fe,Ni)3P, yellowish in color and predominantly found in iron and stony-iron meteorites. Schreibersite can also be found in a variety of other meteorites including some acapulcoites, aubrites, enstatite chondrites and achondrites, lunars, ureilites, winonaites and a smattering of other meteorite types like CM, CO and CB. Schreibersite Click on Term to Read More) grains transiting multiple diamond segments which are now cut by graphite bands (see photos below). Miyahara et al. (2015) found evidence (e.g., nitrogenPrincipal constituent of the Earth’s atmosphere (78.08 vol. % at ground level). Nitrogen is the fifth most abundant element in the universe by atom abundance. Nitrogen comprises only 3.5 vol. % of the atmosphere of Venus and 2.7 vol. % of Mars’s atmosphere. Nitrogen has two isotopes: 14N (99.632 %) and 15N Click on Term to Read More sector zoning) that such large diamonds formed relatively slowly in a melt phase located deep within the UPB. Nabiei et al. (2018) argue that the Al- and Mg-free chromite inclusions present within these large diamonds attest to formation in an iron melt phase void of silicates, such as in a core or melt pod. Importantly, the sulfide + kamacite + schreibersite inclusions present within the diamonds are stable at pressures >20 GPa; such pressures would occur at the center of a Mercury-sized planet, or at the core–mantle boundary of a Mars-sized planet.
click on image for a magnified view
Diagram credit: Nabiei et al., Nature Communications, vol. 9, #1327, pp. 1–9 (2018, open access link)
‘A large planetary body inferred from diamond inclusions in a ureilite meteorite’
(https://doi.org/10.1038/s41467-018-03808-6)
Further analyses of Almahata Sitta specimens led to the discovery of a wide range of xenolithic clasts representing many different chondritic and achondritic lithologies in a manner similar to the structure of the polymict brecciaGeneral term for all breccias that are neither monomict nor dimict. Modified from image source: http://www.saharamet.com/meteorite/gallery/HED/index.html. Kaidun (Bischoff et al., 2010). Evidence exists indicating that all of these clasts came from the Almahata Sitta fall; e.g., detection of short-lived cosmogenic nuclides, very low weathering grade, multiple lithologies among fragments, a high number of rare E-chondriteType of meteorite high in the mineral enstatite and also referred to as E-chondrites. Although they contain substantial amounts of Fe, it is in the form of Ni-Fe metal or sulfide rather than as oxides in silicates. Their highly reduced nature indicates that they formed in an area of the Click on Term to Read More rock types found, diffusionMovement of particles from higher chemical potential to lower chemical potential (chemical potential can in most cases of diffusion be represented by a change in concentration). Diffusion, the spontaneous spreading of matter (particles), heat, or momentum, is one type of transport phenomena. Because diffusion is thermally activated, coefficients for diffusion Click on Term to Read More of PAHs among clasts (Sabbah et al., 2010), and the finding of new and unique meteorite fragments within a small area. The heterogeneous composition of Almahata Sitta could reflect a rubble pile assemblage derived from a catastrophic collision between ureilte and chondritic objects (Kohout et al., 2010; Sánchez and Scheeres, 2014). Alternatively, it is considered that these diverse clasts became gravitationally bound within a common debris disk composed of a disrupted ureilite asteroid, and this disk was then re-accreted into one or more smaller second-generation asteroids. The daughter asteroid(s) became lightly sintered together through subsequent low-energy impacts resulting in a very weak object having a bulk porosity of ~50%.
Another model which better accounts for the various thermal parameters of the ureilite parent body (UPB), as well as the accumulation of a wide variety of exotic lithologies, has been developed (Goodrich et al., 2015; Wilson and Goodrich, 2016). The investigators posit that accretionAccumulation of smaller objects into progressively larger bodies in the solar nebula leading to the eventual formation of asteroids, planetesimals and planets. The earliest accretion of the smallest particles was due to Van der Waals and electromagnetic forces. Further accretion continued by relatively low-velocity collisions of smaller bodies in the Click on Term to Read More of the UPB from ordinary chondrite-like precursor material occurred in the outer asteroid beltBelt located between 2.12 and 3.3 AU from the Sun and located between the orbits of Mars and Jupiter containing the vast majority of asteroids. The asteroid belt is also termed the main asteroid belt or main belt to distinguish it from other asteroid populations in the Solar System such Click on Term to Read More (beyond the ice line) 0.61–0.64 m.y. after CAIsSub-millimeter to centimeter-sized amorphous objects found typically in carbonaceous chondrites and ranging in color from white to greyish white and even light pink. CAIs have occasionally been found in ordinary chondrites, such as the L3.00 chondrite, NWA 8276 (Sara Russell, 2016). CAIs are also known as refractory inclusions since they Click on Term to Read More. Radiogenic heating from 26Al decay led to silicate melting and melt migration to the surface, possibly aided by smelting-generated CO2. After peak temperatures were attained ~4 m.y. after CAIs, a period of slow cooling ensued, during which time gravitational perturbations from large planetary embryos (possibly aided by nebular gas drag) began to influence the UPB orbital mechanics. These forces induced a slow migration sunward over a timescale measured in millions of years, a journey which took the UPB (or possibly a second generation object) inward across the orbital paths of the OC and EC parent bodies. After the severe impact disruption of the primary UPB and re-accretion into one or more daughter bodies—an event dated at 5.4 m.y. after CAIs—the ureilite daughter body (UDB) parental to 2008 TC3 experienced substantial mass accumulation along with large-scale impact gardening. Over time, a deep loosely consolidated regolith was developed on at least a portion of the UDP surface, incorporating a wide variety of foreign impactor material, before it too experienced a disruptive event 20 m.y. ago (based on the 21Ne age of multiple clasts). Ultimately, 2008 TC3 was intercepted by Earth where the complex history of Almahata Sitta could be revealed. Furthermore, it is argued that all of the other ureilite meteorites delivered to Earth also derive from the same UDB, but the exact ejection history for each is still being determined.
Because of the lack of solar-wind-implanted gases in a sample of Almahata Sitta clasts, Bischoff et al. (2010) inferred that the meteorite could not have been derived from a regolith, and it was considered instead to represent a more deeply buried lithology (Hartmann et al., 2011). In contrast, Goodrich et al. (2015) argued that solar gases would only be implanted within the top millimeter of the regolith, and that subsequent gardening may not have accomplished homogenous mixing into deeper regolith layers. Utilizing measurements of gamma-ray emitting radionuclides along with the calculated densityMass of an object divided by its volume. Density is a characteristic property of a substance (rock vs. ice, e.g.). Some substances (like gases) are easily compressible and have different densities depending on how much pressure is exerted upon them. The Sun is composed of compressible gases and is much Click on Term to Read More range of the Almahata Sitta samples, Taricco et al. (2010) determined the shielding depth of ureilite fragment AhS 15 on asteroid 2008 TC3; activity of 60Co and 26Al indicate a shielding depth of 41 (±14) cm.
Results of a trace elementSubstance composed of atoms, each of which has the same atomic number (Z) and chemical properties. The chemical properties of an element are determined by the arrangement of the electrons in the various shells (specified by their quantum number) that surround the nucleus. In a neutral atom, the number of Click on Term to Read More study of a suite of unbrecciated ureilites led Barrat et al. (2016) to propose a revised melting history for the UPB. They concluded that following the segregation of a S-rich metallic coreIn the context of planetary formation, the core is the central region of a large differentiated asteroid, planet or moon and made up of denser materials than the surrounding mantle and crust. For example, the cores of the Earth, the terrestrial planets and differentiated asteroids are rich in metallic iron-nickel. Click on Term to Read More, ureilite precursor material experienced two successive steps of melting and melt extraction. The initial extracted melts were low-density feldspathic lavas containing high abundances of Al, alkalis, and silicaSilicon dioxide, SiO2., comparable to some clasts identified in polymict ureilites and to two trachyandesitic lithologies identified from the Almahata Sitta fall (24.2 g MS-MU-011 [ALM-A] and MS-MU-035). A presumed scenario was presented in which this relatively buoyant silicic liquid was extracted, leaving behind an olivine and pigeoniteLow-Ca clinopyroxene, (Ca,Mg,Fe)SiO3, found as a major mineral in eucrites and shergottites. In order to be considered pigeonite, the clinopyroxene must contain 5 to 20 mol % of calcium (Wo5 - 20). Chondrites of petrologic types 4 and below contain significant low-Ca clinopyroxene. During metamorphism to higher temperatures, all existing residue, and ultimately reached the surface to form a crustOutermost layer of a differentiated planet, asteroid or moon, usually consisting of silicate rock and extending no more than 10s of km from the surface. The term is also applied to icy bodies, in which case it is composed of ices, frozen gases, and accumulated meteoritic material. On Earth, the Click on Term to Read More of some extent. A representative of the next sequential melt to be extracted has not yet been identified among known meteorites, but it is inferred to have been an Al-poor, alkali-depleted liquid possibly more dense than the resulting ureilitic residues. Consequently, this melt material may have been located deeper in the mantleMain silicate-rich zone within a planet between the crust and metallic core. The mantle accounts for 82% of Earth's volume and is composed of silicate minerals rich in Mg. The temperature of the mantle can be as high as 3,700 °C. Heat generated in the core causes convection currents in Click on Term to Read More of the UPB where sampling would be a less likely event. Regardless of the actual density of this second-stage melt, the model scenario infers a partial meltingAn igneous process whereby rocks melt and the resulting magma is comprised of the remaining partially melted rock (sometimes called restite) and a liquid whose composition differs from the original rock. Partial melting occurs because nearly all rocks are made up of different minerals, each of which has a different melting Click on Term to Read More degree of ~17–28% that was sustained throughout the entire melting event, and therefore the mass of this second-stage melt would be proportionately less than that represented by the rare trachyandesitic lithologies. The Al–Mg age determined for the ALM-A trachyandesite sample indicates that the disruption of the ureilite parent body occurred no earlier than ~6.5. m.y. after CAIs (Bischoff et al., 2014).
The ultimate parental source melt of the ureilites was enriched in incompatible trace elements relative to CI chondritesChondrites are the most common meteorites accounting for ~84% of falls. Chondrites are comprised mostly of Fe- and Mg-bearing silicate minerals (found in both chondrules and fine grained matrix), reduced Fe/Ni metal (found in various states like large blebs, small grains and/or even chondrule rims), and various refractory inclusions (such Click on Term to Read More and likely contained phosphate in the form of merrillite, the latter being exhausted along with feldsparAn alumino-silicate mineral containing a solid solution of calcium, sodium and potassium. Over half the Earth’s crust is composed of feldspars and due to their abundance, feldspars are used in the classification of igneous rocks. A more complete explanation can be found on the feldspar group page. Click on Term to Read More prior to the crystallizationPhysical or chemical process or action that results in the formation of regularly-shaped, -sized, and -patterned solid forms known as crystals. Click on Term to Read More of the ureilitic residues. Based on trace element abundances and REEOften abbreviated as “REE”, these 16 elements include (preceded by their atomic numbers): 21 scandium (Sc), 39 Yttrium (Y) and the 14 elements that comprise the lanthanides excluding 61 Promethium, an extremely rare and radioactive element. These elements show closely related geochemical behaviors associated with their filled 4f atomic orbital. Click on Term to Read More patterns among the ureilite samples studied, Barrat et al. (2016) resolved two distinct groups which they termed A and B. The REE abundances for the ureilites constituting the smaller group B are consistent with a fractional melting model in which the critical mass porosity of the residue was zero, thereby leaving no residual melt component following extraction. However, the REE abundances for the ureilites constituting the larger group A are more consistent with a dynamic melting process in which the critical mass porosity of the residue was greater than zero (<2%), resulting in the retention of a critical fraction of melt within the residue.
Exclusive of the primary ureilite components, there was a broad diversity of lithologic types present in 2008 TC3, which constituted <30% of all material recovered. However, since the vast bulk of 2008 TC3 is thought to have been lost as fine dust (≥99.9% of the estimated 42–83 ton pre-atmospheric mass), the asteroid was likely composed primarily of fine-grained, highly-porous, weakly-consolidated ureilitic matrixFine grained primary and silicate-rich material in chondrites that surrounds chondrules, refractory inclusions (like CAIs), breccia clasts and other constituents. Click on Term to Read More material consistent with the reflectance spectra obtained for the asteroid (Goodrich et al., 2015). Examples of some of the diverse samples that have been recovered are listed below (Bischoff et al., 2010, 2015, 2016, 2018; Horstmann and Bischoff, 2010, 2014; Hoffmann et al., 2016; Fioretti et al., 2017):
- ultrafine- to fine-grained ureilites (representing numerous lithologies with varying olivine compositions): MS-185 (ultrafine-grained), MS-MU-001, -018 (high shock, metal–sulfide-rich), -025 (high shock), -027 (high shock), -030 (high shock, metal–sulfide-rich), -032 (high shock, metal–sulfide-rich), -033 (high shock, metal–sulfide-rich), -040 (high shock), -045 (high shock)
- coarse-grained ureilites (representing numerous lithologies with varying olivine compositions: MS-MU-005, -006, -008, -010, -014 (very coarse), -016, -017, -020, -022, -034, -037, -038
- variable grain-sized ureilite breccias: MS-25, -205, -190; MS-MU-004, -021, -028, -042
- highly porous ureilitic (matrix) material: MS-168
- enstatiteA mineral that is composed of Mg-rich pyroxene, MgSiO3. It is the magnesium endmember of the pyroxene silicate mineral series - enstatite (MgSiO3) to ferrosilite (FeSiO3). Click on Term to Read More chondrites (36, representing numerous different enstatite chondrites): EH3 (MS-14), EH4/5 (MS-192, MS-MU-009), EH5 (MS-MU-041, -044), EL3 (MS-1, -17, -177, MS-MU-002, -023, -031), EL3/4 + melt (MS-17, MS-MU-039 [+ melt]), EL3–5 (MS-179), EL4 (MS-MU-029), EL4/5 (MS-192, MS-MU-009), EL5 (MS-196), EL5/6 (MS-7), EL6 (MS-150, MS-MU-007, -015, -024, -026), EL breccias (MS-MU-003), and both EL and EH (MS-155) shock-darkened, impact-melt rocks or impact-melt breccias
- ordinary chondrites: H4 (MS-MU-043), H5 (AhS 25, MS-151 [shock-darkened]), H5/6 (MS-11, with compositional discordance), L4 (AhS A100), LL4/5 (MS-197), H5-an (MS-MU-013)
- unique chondriteChondrites are the most common meteorites accounting for ~84% of falls. Chondrites are comprised mostly of Fe- and Mg-bearing silicate minerals (found in both chondrules and fine grained matrix), reduced Fe/Ni metal (found in various states like large blebs, small grains and/or even chondrule rims), and various refractory inclusions (such Click on Term to Read More: MS-CH, type 3.8 [± 0.1], has petrographic and isotopic affinities to R-chondrites, but is mineralogically anomalous
- Bencubbin-like carbonaceous chondriteCarbonaceous chondrites represent the most primitive rock samples of our solar system. This rare (less than 5% of all meteorite falls) class of meteorites are a time capsule from the earliest days in the formation of our solar system. They are divided into the following compositional groups that, other than Click on Term to Read More: MS-181, a 58.6 g chondrule-like clastA mineral or rock fragment embedded in another rock. Click on Term to Read More containing metalElement that readily forms cations and has metallic bonds; sometimes said to be similar to a cation in a cloud of electrons. The metals are one of the three groups of elements as distinguished by their ionization and bonding properties, along with the metalloids and nonmetals. A diagonal line drawn Click on Term to Read More globules and silicates in a 60:40 ratio, having an O-isotopic composition consistent with bencubbinites
- C2 carbonaceous chondrite: AhS 202 (photo; Fioretti et al., 2017, #1846)
- C1 carbonaceous chondrite: AhS 91/91A and 671 (photo; Goodrich et al., 2018, #1321)
- niningerite-bearing, fine-grained ureilitic fragment (linking E chondrites): MS-20
- sulfide-metal assemblage in a fine-grained ureilitic fragment: MS-158, -166
- ungroupedModifying term used to describe meteorites that are mineralogically and/or chemically unique and defy classification into the group or sub-group they most closely resemble. Some examples include Ungrouped Achondrite (achondrite-ung), Ungrouped Chondrite (chondrite-ung), Ungrouped Iron (iron-ung), and Ungrouped Carbonaceous (C-ung). Click on Term to Read More enstatite- and metal-rich achondriteAn achondrite is a type of stony meteorite whose precursor was of chondritic origin and experienced metamorphic and igneous processes. They have a planetary or differentiated asteroidal origin where the chondritic parent body reached a sufficient size that through heating due to radioactive decay of 26Al (aluminum isotope) and gravitational Click on Term to Read More fragments: MS-MU-019 (characteristics similar to NWA 8173/10271); MS-MU-036 (similar to MS-MU-019 and Itqiy [Bischoff et al., 2016]); AhS 38 (similar to MS-MU-019 and Itqiy but contains olivine [Goodrich et al., 2018]); AhS 60 (possible E IMR analogous to Portales Valley [Goodrich et al., 2018])
- the first known plagioclase-bearing olivine–augiteHigh-Ca clinopyroxene, (Ca,Mg,Fe)SiO3, that occurs in many igneous rocks, particularly those of basaltic composition. In order to be considered augite, the clinopyroxene must contain 20 to 45 mol % of calcium (Wo20 - 45). An important and unique Martian meteorite is NWA 8159, that has been classified as an augite basalt. Click on Term to Read More ureilite lithology: MS-MU-012
- trachyandesitic clasts: 1) MS-MU-011 (view 1), MS-MU-011 (view 2), MS-MU-011 (sample ALM-A); plagioclase-enriched (~70 vol%) with pockets of gemmy olivine (see photos below), likely sampling the UPB crust (or possibly an alkali- and water-rich localized melt pocket); calculated Ar–Ar age of ~4.556 b.y. and Pb–Pb age of ~4.562 b.y. (Bischoff et al., 2013, 2014; Delaney et al., 2015; Turrin et al., 2015; Amelin et al., 2015); 2) MS-MU-035; anorthoclase and/or plagioclase-enriched (~65 vol%) (Bischoff et al., 2016)
MS-MU-011—trachyandesite clast with gemmy olivines
click on image for a magnified view
Photos courtesy of Stephan Decker–Meteorite Shop and Museum
A consortium was established to study Alamahta Sitta and much has since been learned. This meteorite is a characteristic ureilite that has experienced high degrees of silicate partial melting. It underwent rapid cooling (0.05–2°C/hour) and experienced reductionOxidation and reduction together are called redox (reduction and oxidation) and generally characterized by the transfer of electrons between chemical species, like molecules, atoms or ions, where one species undergoes oxidation, a loss of electrons, while another species undergoes reduction, a gain of electrons. This transfer of electrons between reactants Click on Term to Read More following a sudden pressure loss, possibly due to parent body disruption and re-accretion into a second generation of objects 10–100 m in diameter (Herrin et al., 2010). Large carbonaceous grains are present, which consist mostly of graphite and contain extraterrestrial two- to six-carbon aliphatic amino acids interspersed with a distinct range of polycyclic aromatic hydrocarbons (PAHs) (Callahan et al., 2009; Zare et al., 2009). The presence of various amino acidA small molecule containing and amine group (–NH2) at one end and a carboxylic acid group (–COOH) at the other. They link up with various other amino acids to form proteins. Because they are used to build proteins, most of the human body consists of amino acids with their abundance second Click on Term to Read More decomposition products attests to thermal alteration of organicPertaining to C-containing compounds. Organic compounds can be formed by both biological and non-biological (abiotic) processes. Click on Term to Read More compounds on the parent asteroid.
Fitting the measured radionuclideRadioactive isotope - Atomic nuclide that decays radioactively . Click on Term to Read More concentrations to the known size of the pre-atmospheric asteroid for a range of estimated densitiesMass of an object divided by its volume. Density is a characteristic property of a substance (rock vs. ice, e.g.). Some substances (like gases) are easily compressible and have different densities depending on how much pressure is exerted upon them. The Sun is composed of compressible gases and is much Click on Term to Read More, and then utilizing the average grain density for ureilites, the total porosity of the 2008 TC3 asteroid was determined to be ~55%, consistent with its early disruption upon atmospheric entry (Welten et al., 2010). Notably, the bulk density (~1.8 g/cm³) of the asteroid was lower than that of the recovered meteorites (1.77–3.26 g/cm³). Given the density of Alamahta Sitta, the pre-atmospheric size of the asteroid was calculated to have been ~4.1 m in diameter with a volume of 28 m³, and it had an oblong shape with a total mass of 51,000 kg.
In a study of oxygenElement that makes up 20.95 vol. % of the Earth's atmosphere at ground level, 89 wt. % of seawater and 46.6 wt. % (94 vol. %) of Earth's crust. It appears to be the third most abundant element in the universe (after H and He), but has an abundance only Click on Term to Read More isotopes for Alamahta Sitta, Rumble III et al. (2010) established an oxygen three-isotope plot for a small lot of fragments. As with typical ureiltes, the values are heterogeneous and plot in the upper one-third of the carbonaceous chondrite anhydrous mineralInorganic substance that is (1) naturally occurring (but does not have a biologic or man-made origin) and formed by physical (not biological) forces with a (2) defined chemical composition of limited variation, has a (3) distinctive set of of physical properties including being a solid, and has a (4) homogeneous Click on Term to Read More (CCAM) trend line, spanning the entire compositional range of known monomict and polymict ureilites (Rumble III et al., 2010). These data are consistent with the hypothesis that ureilites are derived from a minimally molten (20–30%) carbonaceous chondrite body, and that ureilites spanning the entire O-isotopic range constituted a common parent body. Based on its O-isotopic signature and major element chemistry, Rai et al. (2016) calculated that the precursor material for the UPB had a mixed composition consisting of CI, CM, EH, and Mg-rich chondrules.
Image credit: Rai et al., 47th LPSC, #1702 (2015)
By contrast, studies of ε54Cr anomalies in Alamahta Sitta samples by Qin et al. (2010) revealed a negative anomaly that is consistent only with ordinary chondrites and achondrites such as HED members, but not with carbonaceous chondrites which have a positive anomaly. The ε54Cr value infers that Alamahta Sitta, and possibly all ureilites, were not derived from a carbonaceous chondrite parent body as commonly presumed. Valdes et al. (2016) studied the Ca-isotopic signature for ureilites, which serves as an excellent tool for resolving genetic relationships, and found a wide range in δ44Ca values unique from values for carbonaceous chondrites or any other chondritic material. Goodrich et al. (2015) recognized that the Cr, Ti, and Ni isotopic compositions of ureilites are more consistent with an origin on an OC or EC parent body than a CC parent body. They also showed that the inferred lithophile elementElement that tends to be concentrated in the silicate phase, e.g., B, O, halogens, alkali earths, alkali metals, Al, Si, Sc, Ti, V, Cr, Mn, Y, Zr, Nb, REE, Hf, Ta, W, Th, and U. composition of ureilite precursor material was most similar to OC and R-chondrites. In a comparison of Si/Mg vs. Mg/Mn (see below), the ureilites plot near OC and R-chondrites rather than CC or E-chondrites.
Image credit: Goodrich et al., MAPS, vol. 50, #4, p. 792 (2015)
‘Origin and history of ureilitic material in the solar systemThe Sun and set of objects orbiting around it including planets and their moons and rings, asteroids, comets, and meteoroids.: The view from asteroid 2008 TC3 and the Almahata Sitta meteorite’
(http://dx.doi.org/10.1111/maps.12401)
Warren (2011) determined that the isotopeOne of two or more atoms with the same atomic number (Z), but different mass (A). For example, hydrogen has three isotopes: 1H, 2H (deuterium), and 3H (tritium). Different isotopes of a given element have different numbers of neutrons in the nucleus. Click on Term to Read More signatures of Δ17O, ε54Cr, ε50Ti, and ε62Ni can be utilized to resolve carbonaceous from non-carbonaceous meteorites; the carbonaceous meteorites have positive values for all of these elements, while the non-carbonaceous meteorites have negative values. An example coupled Δ17O vs. diagram is shown below to demonstrate the separation between carbonaceous and non-carbonaceous meteorites. Ureilites plot in the non-carbonaceous field furthest removed from the carbonaceous field.
Diagram credit: Sanders et al., MAPS, vol. 52, #4, p. 695 (2017)
‘Origin of mass-independent oxygen isotope variation among ureilites: Clues from chondrites and primitive achondrites’
(http://dx.doi.org/10.1111/maps.12820)
In a study conducted by Srinivasan et al. (2018), it was determined that the O-isotopic composition and Al–Mg age data are reasonably consistent among several different achondrites: the ungrouped NWA 11119 and NWA 7325, and the trachyandesite clasts in the Almahatta Sitta anomalous polymict ureilite (MS-MU-011/ALM-A and MS-MU-035). In addition, some notable geochemical similarities have been identified. Moreover, it was recognized that these are the only achondrites known that plot on the carbonaceous chondrite anhydrous mineral (CCAM) line at the high-δ18O end. Although these meteorites do have distinct Fe/Mn ratios, the range in values is much less than that within the acapulcoite–lodraniteRare type of primitive achondrite named after the Lodran meteorite that fell in Pakistan in 1868. Initially, lodranites were grouped with the stony-iron meteorites because they contain silicates (olivine, orthopyroxene, and minor plagioclase) and Fe-Ni metal in nearly equal proportions. However, since discovery of the closely related acapulcoite group, lodranites Click on Term to Read More clan, the latter of which is known to represent a single parent body. The many similarities that have been documented among these diverse meteorites suggest that a genetic relationship (i.e., a common differentiated parent body) may exist (see diagram below).
Oxygen Three-Isotope Plot
Diagram credit: Srinivasan et al., Nature Communications, vol. 9, p. 4 (2018, open access link)
‘Silica-rich volcanism in the early solar systemDefinable part of the universe that can be open, closed, or isolated. An open system exchanges both matter and energy with its surroundings. A closed system can only exchange energy with its surroundings; it has walls through which heat can pass. An isolated system cannot exchange energy or matter with dated at 4.565 Ga’
(https://doi.org/10.1038/s41467-018-05501-0)
A stone recovered from the Alamahta Sitta strewn fieldArea on the surface containing meteorites and fragments from a single fall. Also applied to the area covered by tektites, which are produced by large meteorite impacts. Strewnfields are often oval-shaped with the largest specimens found at one end. Given that the largest specimens go the greatest distance, a meteoroid's, designated AhS 91/91A (and a similar stone AhS 671), is a breccia composed of ureilitic material in contact with a dominant C1 lithology; this material possibly represents the bulk matrix of the 2008 TC3 asteroid (Goodrich et al., 2017 [#6214). Contrary to the negative Cr-isotopic values obtained from all ureilites analyzed thus far (ε54Cr ~ –0.90), the C1 lithology has the highest ε54Cr composition measured for any meteorite (ε54Cr +1.83 [±0.08]; Sanborn et al., 2017, #6277) (see top diagram below). The bulk mineralogy of AhS 91A was determined to be most similar to C1 carbonaceous chondrite material, but is unlike any known carbonaceous chondrite group represented in our collections (Yin et al., 2018 [#1810]). This C1 lithology is intimately mixed with minerals compositionally and texturally consistent with ureilites (olivine + pigeonite + albite [= polymict ureilite ‘albitic lithology’ and AhS trachyandesite MS-MU-011/035]), along with chondrulesRoughly spherical aggregate of coarse crystals formed from the rapid cooling and solidification of a melt at ~1400 ° C. Large numbers of chondrules are found in all chondrites except for the CI group of carbonaceous chondrites. Chondrules are typically 0.5-2 mm in diameter and are usually composed of olivine Click on Term to Read More and metal derived from ordinary and enstatite chondrites. An O-isotopic analysis shows that the C1 material from AhS 91 and 671 plot along the CCAM line in a unique location (see bottom diagram below); notably, the C2 clast AhS 202 plots within the ureilite field.
Chromium vs. Oxygen Isotope Plot
Diagram credit: Yin et al., 49th LPSC, #1810 (2018)
Oxygen Isotope Plot
Diagram credit: Goodrich et al., 49th LPSC, #1321 (2018)
It was previously considered by some that the observed correlations between oxygen isotopes and redoxOxidation and reduction together are called redox (reduction and oxidation) and generally characterized by the transfer of electrons between chemical species, like molecules, atoms or ions, where one species undergoes oxidation, a loss of electrons, while another species undergoes reduction, a gain of electrons. This transfer of electrons between reactants Click on Term to Read More trends in ureilites was inherited from accreted material which previously had undergone high-temperature solid–gas exchange in the nebulaAn immense interstellar, diffuse cloud of gas and dust from which a central star and surrounding planets and planetesimals condense and accrete. The properties of nebulae vary enormously and depend on their composition as well as the environment in which they are situated. Emission nebula are powered by young, massive Click on Term to Read More. Extensive smelting has also been invoked to explain these correlations. However, Sanders et al. (2017) argue that the positive correlation observed between Fa content in olivine and Δ17O, as well as that observed for other O-isotopic and redox trends (e.g., the plot of oxygen isotope ratios along a mass-independent [slope ~1] line; the precise correlation between FeO/MgO and FeO/MnO molar ratios), also exists in equilibrated ordinary and R chondrites. However, studies have shown that these same trends that exist among ordinary chondriteWork in Progress Ordinary chondrites (OCs) are the largest meteorite clan, comprising approximately 87% of the global collection and 78% of all falls (Meteoritical Society database 2018)1. Meteorites & the Early Solar System: page 581 section 6.1 OC of type 5 or 6 with an apparent shock stage of S1, Click on Term to Read More clan meteorites were not derived through accretion of primary nebula material, but instead were the result of varying degrees of secondary low-temperature aqueous alteration on their respective parent bodies (R > LL > L > H > low-FeO chondrites). This alteration process involved oxidationOxidation and reduction together are called redox (reduction and oxidation) and generally characterized by the transfer of electrons between chemical species, like molecules, atoms or ions, where one species undergoes oxidation, a loss of electrons, while another species undergoes reduction, a gain of electrons. This transfer of electrons between reactants Click on Term to Read More of metal by isotopically heavy ice/water (Δ17O possibly as high as +20‰) to form FeO that was subsequently incorporated into existing silicates to establish the correlated redox trends. Therefore, Sanders et al. (2017) infer that a similar aqueous alteration process, induced by primary accreted water ice, occurred during the early history (pre-disruption) of the chondritic parent body from which the ureilites would ultimately derive. They propose that regions of the ureilite parent body with abundant accreted metal reached a maximum oxidation state with an olivine composition of ~Fa25, while other regions with less accreted metal experienced much less oxidation which is reflected in olivine of ~Fa5.
Chromium isotopic compositions were studied for Alamahta Sitta and two other polymict ureilites by Qin et al. (2010), and a similar isochron was found for all samples corresponding to an absolute age of 4.5636 (±0.0022) b.y., with reference to the angriteType of evolved achondrite meteorite that represent some of the earliest stages of asteroidal differentiation and magmatism in our solar system. Angrites are named for the Angra dos Reis meteorite, which fell in Rio de Janeiro, Brazil, in early 1869. They are basaltic (mafic) rocks, often containing porous areas, and Click on Term to Read More D’Orbigny. It was shown that the ε54Cr systematics for Alamahta Sitta and other ureilites are unlike those from any of the carbonaceous chondrite groups, attesting to their origin on distinct parent bodies. It is noteworthy that the ε54Cr systematics for Alamahta Sitta are very similar to those of the HED parent body, and it can be inferred that both the ureilite and HED parent bodies accreted and experienced igneous melting very early in solar system history—within 5 m.y. of CAISub-millimeter to centimeter-sized amorphous objects found typically in carbonaceous chondrites and ranging in color from white to greyish white and even light pink. CAIs have occasionally been found in ordinary chondrites, such as the L3.00 chondrite, NWA 8276 (Sara Russell, 2016). CAIs are also known as refractory inclusions since they Click on Term to Read More formation—in a similar region of the solar nebula. The short-lived Hf–W chronometer was applied to a large number of ureilites to constrain the timing of metal–silicate separation on the UPB (Budde et al., 2015). Their calculations indicate that parent body differentiationA process by which a generally homogeneous chondritic body containing mostly metal, silicates and sulfides will melt and form distinct (differentiated) layers of different densities. When the melting process continues for a long enough period of time, the once chondritic body will re-partition into layers of different composition including Click on Term to Read More occurred as early as 2.6 (±0.9) m.y. after CAI formation. As a consequence of this timing and in accord with thermal history modeling, accretion of the UPB would have occurred ~1.4 m.y. after CAIs. In further studies based on Al–Mg systematics, Hublet et al. (2016) obtained a model age for differentiation on the UPB of 1.09 (±0.75) m.y. after CAIs, indicating that accretion began even earlier in solar system history.
A classification of the Alamahta Sitta polymict breccia ureilite was given by Zolensky et al. (2010). They concluded that its Mg# characterizes it as a member of Berkley’s Group II, but that it falls at the extreme Mg-rich range; however, other fragments studied revealed a ferroan composition (Mikouchi et al., 2010). In the scheme of Goodrich et al. (2004), in which ureilites are divided into three distinct subgroups—olivine–pigeonite, olivine–orthopyroxeneOrthorhombic, low-Ca pyroxene common in chondrites. Its compositional range runs from all Mg-rich enstatite, MgSiO3 to Fe-rich ferrosilite, FeSiO3. These end-members form an almost complete solid solution where Mg2+ substitutes for Fe2+ up to about 90 mol. % and Ca substitutes no more than ~5 mol. % (higher Ca2+ contents occur Click on Term to Read More, and augite-bearing—Alamahta Sitta is a partial melt residue best described as a pigeonite-olivine ureilite due to its greater abundance of pigeonite over olivine. Unusually, due to its low Fe content, Alamahta Sitta plots among the olivine-orthopyroxene subgroup. In contrast, 39 IR spectra taken from 26 stones resulted in a heterogeneous profile of silicates ranging from nearly pure olivine to nearly pure pyroxeneA class of silicate (SiO3) minerals that form a solid solution between iron and magnesium and can contain up to 50% calcium. Pyroxenes are important rock forming minerals and critical to understanding igneous processes. For more detailed information, please read the Pyroxene Group article found in the Meteoritics & Classification category. Click on Term to Read More (pigeonite), while a combined mass weighted average of all of the spectra resulted in an olivine:pyroxene ratio of 74:26, well within the range of the spectra from other ureilites (Sandford et al., 2010). The fine-grained lithology in Almahata Sitta shows evidence of reduction through impact smelting accompanied by abundant release of CO2 gas, and shock granulation of both olivine and pyroxene. This reduction process occurred at the time of the catastrophic disruption of the UPB during peak temperatures which resulted in the production of interstitial silica and tiny Fe-metal particles (Warren and Rubin, 2010); a rapid cooling phase followed.
The average CRE age based on 21Ne for four Alamahta Sitta ureilite fragments was determined to be 14.5 (±0.9) m.y. (Welten et al., 2010, 2011). This age falls well within the range of typical ureilites and represents the time since asteroid 2008 TC3 broke away from its larger parent asteroid. A more reliable method employed by Welten et al. (2010) gave an average CRE age of 19.5 (±2.5) m.y., which is consistent with other ureilites. Further noble gasElement occurring in the right-most column of the periodic table; also called "inert" gases. In these gases, the outer electron shell is completely filled, making them very unreactive. Click on Term to Read More studies of ureilite fragments conducted by Nagao et al. (2014) gave similar 3He- and 21Ne-based CRE ages of ~20 m.y. The He-, Ne-, and Ar-based CRE age values of H and L chondriteOrdinary chondrites low in free Ni-Fe metal (4 to 10 vol. %), containing olivine (Fa22-26) and the orthopyroxene hypersthene (Fs19-22). Average chondrule diameters (0.7 mm) are larger than those in H chondrites. The asteroid 433 Eros is suspected as a parent body, based on reflectance spectra, but most L chondrites Click on Term to Read More material from Alamahta Sitta were determined by Meier et al. (2010) to be ~13.6–27 m.y., overlapping within error those of Alamahta Sitta ureilites. Additional noble gas studies conducted by Riebe et al. (2014) of several chondritic samples from Almahata Sitta (E, LL, CB, R-like chondrites) also support this age. With inclusion of all CRE age results obtained to date, they derived an average age of 19.7 (±2.8) m.y. This relatively young CRE age for the various components of 2008 TC3 indicates that it was located in close proximity to a mean-motion resonance. The gas retention age of the L chondrite samples from Almahata Sitta is distinct from that of the typical L group members, the latter forming an ~500 m.y. age cluster. Notably, one E chondrite sample studied by Riebe et al. (2017) designated MS-179, which is linked to the Almahata Sitta fall through multiple lines of evidence, presents a younger CRE age of 11 (±1.4) m.y. They suggest this lower CRE age might represent the actual age of the 2008 TC3 meteoroid as an independent object in space subjected to galactic cosmic raysHigh-energy subatomic particles mainly originating outside the Solar System that continuously bombard the Earth from all directions. They represent one of the few direct samples of matter from outside our solar system and travel through space at nearly the speed of light. These charged particles – positively charged protons or Click on Term to Read More (4π exposure), while the older ages of the other components could be attributed to pre-irradiation (2π exposure) within a regolith setting on the parent asteroid. Further noble gas data were obtained for the C1 breccia clast AhS 91A by Riebe et al. (2018). They found that it has a significantly lower CRE age (as low as ~8 m.y) than other Almahata Sitta components measured to date; however, like all other AhS samples, and consistent with its low CRE age indicating residence at some depth (at least a few meters) within a regolith, no solar-wind-implanted noble gases were observed.
In an attempt to identify possible common ejection events among the ureilites, Beard and Swindle (2017) conducted a comparative study of 39 different samples utilizing three parameters: CRE age, Fo content in olivine (Mg#), and Δ17O value. They resolved ten potential clusters, several of which show concordance in their CRE age and Mg# but not in Δ17O value (heterogeneous), and three that are concordant in all three parameters (homogeneous). The oldest cluster they resolved, comprising DaG 084, DaG 319, Goalpara, and Haverö (homogeneous, although the Δ17O values for DaG 084 and DaG 319 have not yet been determined), reflects an ejection event that occurred 20.1 (±1.2) m.y. ago. The CRE age of this cluster is consistent with the average of all CRE age results obtained to date (see Riebe et al. [2017] information above). However, Beard and Swindle (2017) utilized alternate data for Almahata Sitta which provided a CRE age of 15.80 m.y., and they have included it along with GRA 98032 and Lahrauli within a heterogeneous cluster dated at 15.5 (±0.3) m.y.
Trapped noble gas contents and Xe-isotopic compositions in Alamahta Sitta are consistent with those of other ureilites (Ott et al., 2010), with sub-µm- to several µm-sized diamonds serving as the main noble gas carrier (Murty et al., 2010). The U,Th–He ages of chondrite clasts of ~3.8 b.y. may represent the time of their incorporation into the ureilite host, which seems to correspond with the Late Heavy BombardmentPeriod between ~4.0 to 3.8 Ga ago when the Moon and other objects in the Solar System were pounded heavily by wayward asteroids. The evidence for the Late Heavy Bombardment (LHB) includes the lunar maria basins and similar structures elsewhere, such as the Caloris Basin on Mercury and the great Click on Term to Read More period (Welten et al., 2011).
The magnetic signature of Alamahta Sitta was found to be identical to that of previously studied ureilite falls, containing kamacite (two distinct phases), suessite (an iron silicide formed in situ from pre-existing ureilitic metal under low pressure [regolith] and very low redox conditions; Downes et al., 2010), schreibersite, and troiliteBrass colored non-magnetic mineral of iron sulfide, FeS, found in a variety of meteorites. Click on Term to Read More, as well as a daubreelite-heideite phase new to ureilites which is possibly a contaminate from xenolithic clasts (Hoffmann et al., 2010). Ordinary and enstatite chondriteType of meteorite high in the mineral enstatite and also referred to as E-chondrites. Although they contain substantial amounts of Fe, it is in the form of Ni-Fe metal or sulfide rather than as oxides in silicates. Their highly reduced nature indicates that they formed in an area of the Click on Term to Read More xenolithic clasts tend to have a much higher magnetic susceptibility. Hochleitner et al. (2010) investigated the mineralogy of Almahata Sitta and discovered up to five distinct kamacite phases. The metal phase (Kamacite I) that forms large sheets between silicate grains has a unique composition, and it was likely introduced through the impact of a Ni-poor iron meteoriteIron meteorites consist mostly of metallic iron alloyed with typically between ~5 to ~30 wt% nickel. The main metal phases are kamacite α-(Fe, Ni) and taenite y-(Fe, Ni). Based on their group classification, they may also contain a small weight percentage of one or more of the following minerals: • Click on Term to Read More. Troilite blebs located mainly in carbon-rich veins are thought to be the result of olivine reduction processes. As a result of shock re-melting processes in Almahata Sitta, grain boundary FeNi-metal particles are complex assemblages not observed in main group ureilites, comprising variable combinations of taeniteLess common than kamacite, both taenite and kamacite are Ni-Fe alloys found in iron meteorites. Taenite, γ-(Fe,Ni), has 27-65 wt% Ni, and forms small crystals that appear as highly reflecting thin ribbons on the etched surface of a meteorite; the name derives from the Greek word for "ribbon." Click on Term to Read More, kamacite, Fe-carbide (coheniteFe-Ni-Co carbide, (Fe,Ni,Co)3C, that occurs as an accessory constituent in several iron meteorites, and coarse octahedrites with < 7 wt. % Ni. Click on Term to Read More), Fe-phosphide (scheibersite), and Fe-sulfide (Aoyagi et al., 2013). Mikouchi et al. (2014) identified an Fe-martensite phase in Almahata Sitta that is a product of very rapid quenching (>1°C/sec) at relatively low temperatures (~300–500°C). They hypothesized that the martensite was formed through an impact-related heating and fragmentation event on a small ureilitic asteroid; the asteroid probably represents a re-accreted daughter object following the collisional disruption of the original ureilite parent body.
Spectral studies of the many asteroid families have revealed some potential matches to 2008 TC3 and Almahata Sitta, especially the F-class Nysa-Polana family located in the inner Main BeltBelt located between 2.12 and 3.3 AU from the Sun and located between the orbits of Mars and Jupiter containing the vast majority of asteroids. The asteroid belt is also termed the main asteroid belt or main belt to distinguish it from other asteroid populations in the Solar System such Click on Term to Read More near the 3:1 resonance. The Nysa-Polana family has a low orbital inclinationAngle between the plane of an object's orbit and the ecliptic; the inclination of a moon's orbit is the angle between the plane of its orbit and the plane of its primary's equator. Click on Term to Read More similar to that observed for 2008 TC3 along its trajectory to Earth (Gayon-Markt, 2011). This asteroid family contains three different types of material, including rare primitive B-type asteroids akin to ureilites, and thermally altered stony S-type and intermediate X-type asteroids. Spectrally, each of these three asteroid types have been identified in the Almahata Sitta fragments, suggesting that this mixture of asteroid types was the result of multiple low-velocity collisions. Another possible asteroid match is the Hoffmeister family located near the 5:2 resonance (Welten et al, 2010). There is also a possibility that the foreign clasts in Almahata Sitta were derived from a collision with the S-class Mildred family located in the inner Main Belt (Jenniskens et al, 2010).
As a quickly recovered fall, Almahata Sitta has a weathering grade of W0. It has a shock stageA petrographic assessment, using features observed in minerals grains, of the degree to which a meteorite has undergone shock metamorphism. The highest stage observed in 25% of the indicator grains is used to determine the stage. Also called "shock level". Click on Term to Read More of S0, which is consistent with its low abundance of nanodiamonds, but inconsistent with the granoblastic, mosaicized textures observed in olivine and pyroxene (Mikouchi et al., 2010). The specimen of Alamahta Sitta shown above is a 0.33 g crusted partslice sectioned from an undesignated coarse-grained lithology, which represents the most abundant lithology recovered (Horstmann and Bischoff, 2014).
Ureilites Are Finally Figured Out
Image credit: Goodrich et al., MAPS, vol. 50, #4, p. 784 (2015)
‘Origin and history of ureilitic material in the solar system: The view from asteroid 2008 TC3 and the Almahata Sitta meteorite’
(http://dx.doi.org/10.1111/maps.12401)
Special thanks to Siegfried Haberer and Stephan Decker for providing specimens of this special meteorite and many of its xenolithic inclusions to the scientific and collector communities.